含碳气溶胶源解析
Information for the paper
Title: Source apportionment of carbonaceous aerosols in Beijing with radiocarbon and organic tracers: insight into the differences between urban and rural sites
Author: Hou Siqi
Year: 2021
Journal: Atmospheric Chemistry and Physics
URL: https://acp.copernicus.org/articles/21/8273/2021/
Introduction
- The total content of carbonaceous aerosols (total carbon, TC) can be divided into organic carbon (OC) and elemental carbon (EC) according to their physical, chemical, and optical properties.
- Radiocarbon (14C) analysis is a powerful tool for the quantification of fossil and non-fossil contributions to carbonaceous aerosols, as non-fossil sources contain a high contemporary 14C content, while the fossil fractions are free of 14C.
- Moreover, clear seasonal trends of non-fossil and fossil source contributions to water-insoluble OC (WINSOC) and water-soluble OC (WSOC) were found. Non-fossil sources were the major contributor (59%) to WINSOC in summer and autumn, whereas fossil fuel emissions were predominant in winter and spring (Liu et al., 2013).
- However, 14C measurements do not permit direct discrimination of specific sources (e.g. biomass burning or secondary OC, SOC) of modern carbon.
- 14C analysis combined with AMS-PMF (positive matrix factorization analysis of data from an online Aerodyne aerosol mass spectrometer) data has contributed to the identification of sources from primary emissions and secondary formation.
- The Gelencsér method provides a first-order source apportionment of organic aerosol from fossil fuel combustion, biomass burning, biogenic emissions, and secondary organic aerosol, using measurements of specific organic tracers emitted by fossil and non-fossil sources and their OC/EC ratios derived from literature (Gelencsér et al., 2007)
- This method was derived in a European context, but for China the inclusion of food cooking and coal combustion is required. Based upon this consideration, an extended Gelencsér (EG) method that includes quantification of fossil and contemporary EC and OC by 14C analysis has been developed in this study.
- As levoglucosan (1,6-anhydro-β-Dglucopyranose, LG) is an almost specific biomass burning tracer as the main pyrolysis product from cellulose (Puxbaum et al., 2007; Simoneit et al., 1999), concentrations of OC from biomass burning can be obtained by multiplying LG with suitable OC/LG ratios (Gelencsér et al., 2007; Zdrahal et al., 2002).
Methodology
- PM2.5 concentrations on 22 November and 1 December at IAP and PG sites were lower than 75 μg/m3 and regarded as non-haze air days, in contrast to other wintertime samples collected during haze pollution days.
- The 14C of TC and WINSTC was measured using a one-step protocol under pure O2 (99.9995 %) at 760 ℃ for 400 s using an elemental analyser coupled with the accelerator mass spectrometer Mini Carbon Dating System (MICADAS) at the Laboratory for the Analysis of Radiocarbon (LARA; University of Bern).
- The methodology to determine biomass burning tracers, including levoglucosan, mannosan, and galactosan, is described elsewhere (Fu et al., 2016).
Results and discussion
Overall results
- The average concentrations of PM2.5 were 91.2±63.7 and 99.7±77.8 μg/m3 at the IAP and PG sites in winter, respectively.
- Organic carbon and elemental carbon (OC and EC) are important constituents of PM2.5, accounting for 30.9±9.3% and 43.6±17.9% of PM2.5 mass at the IAP and PG sites in winter.
- The average EC concentrations for 14C analysis varied from 3.8±2.1 μg/m3 (IAP) and 5.4±2.6 μg/m3 (PG) in winter for the urban and rural sites, respectively.
- The mass concentration of OC for 14C analysis was 4.1–44.9 and 12.1–85.0 μg/m3 at IAP and PG in winter.
- The OC/EC ratios at IAP and PG were in the range of 4.1– 14.9 and 6.2–14.6 in winter, which were all higher than 2.0 or 1.1, suggesting an important contribution from secondary organic carbon (SOC).
- ECf refers to EC from coal combustion and liquid fossil fuel (i.e., mainly vehicle emissions) and ECnf from biomass burning. As shown in Fig. 2, high concentrations of ECf and ECnf were found in both urban and rural sites in winter, suggesting elevated emissions from primary fossil and non-fossil sources like coal combustion and biomass burning. Residential coal consumption and biomass burning are still important in winter, especially in rural areas, due to intensive heating activities in the cold season.
- OC from fossil sources (OCf) arises mainly from coal combustion and vehicle emissions, while OC from non-fossil sources (OCnf) comes mainly from biomass burning, biogenic emissions, and cooking.
- ECf contributed 7.6±2.1% and 6.0±1.4% to TC at the IAP and PG sites in winter. Higher contributions of ECf were found on the polluted days in wintertime, showing that the PM2.5 concentrations may be elevated due to direct emission from coal combustion and vehicle exhaust.
- Among the four fractions, WINSOCf had the highest contribution to TC in winter (37.9±4.5% at IAP and 36.2±4.7% at PG), followed by WSOCf (22.1±5.2 %) at IAP and WINSOCnf (20.8±3.4 %) at PG.
- Moreover, WINSOC was dominated by fossil sources at both sites in winter (IAP: 72.6±3.6%; PG: 63.4±6.5%), while the non-fossil fractions significantly increased from winter to summer.
- Even though WINSOCnf and WSOCnf cannot be attributed specifically to biomass burning, biogenic emissions, cooking, or secondary formation, it is likely that biogenic-derived POC and SOC make a pronounced contribution.
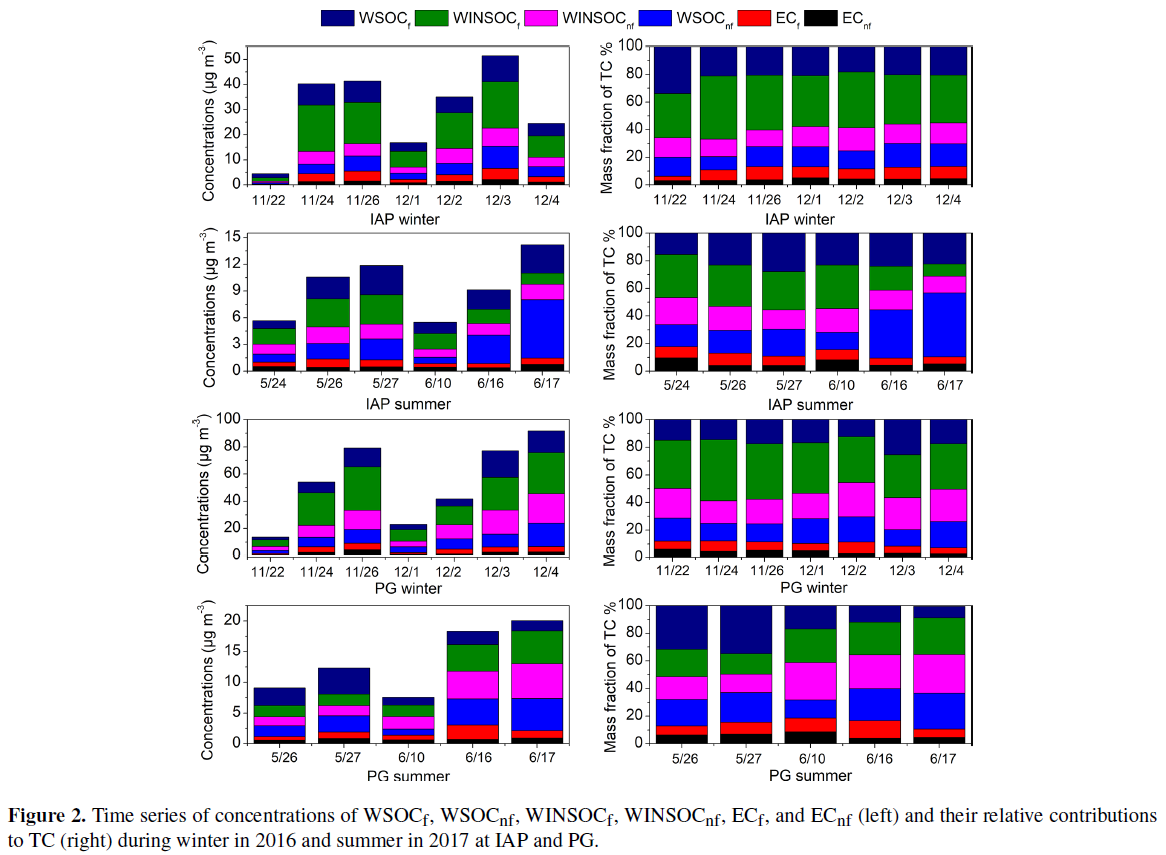
Source apportionment by an extended Gelencsér method
- Much higher concentrations of LG were observed in the winter (311±193 ng/m3 at IAP and 634±483 ng/m3 at PG) than those in the summer (27.9±29.6 ng/m3 at IAP and 74.0±34.2 ng/m3 at PG). In addition, LG concentrations at the rural site were higher than those at the urban site in both winter and summer.
- During winter, LG correlated well with PM2.5, OC, and EC at PG, with correlation coefficients of 0.89, 0.89, and 0.81, respectively. These are higher than those at IAP (correlation coefficients of 0.56, 0.60, and 0.74, respectively), suggesting a more significant influence of biomass burning upon PM2.5 in PG.
- During the wintertime, the increasing use of biofuel for heating exacerbates the biomass pollution, and the stable atmospheric conditions also enhance the accumulation of LG.
- Compared with widely used cleaner fossil energy and renewable energy available in urban areas, rural households are still largely using straw and wood for cooking and heating. Moreover, open burning of crop residues during the post-harvest months (May to July and October to November in North China) in rural areas is still frequently performed in spite of prohibition by the government.
- The water-soluble potassium ion (K+) has been used as a biomass burning tracer previously, due to its good relationship with LG. However, in this study, the Pearson correlation coefficients of K+ with LG are 0.51 and 0.86 in winter and 0.85 and 0.51 in summer for IAP and PG, indicating other non-biomass sources of K+. Indeed, the sources of K+ in the atmosphere are diverse, including sea salt, cooking, dust, coal combustion, and waste incineration, which makes K+ less suitable as a biomass burning tracer (Zhang, et al., 2010).
- The ratios of levoglucosan to mannosan (LG/MN) and to galactosan (LG/GA) can help us to infer the (OC/LG)bb and (EC/OC)bb ratios from certain types of biomass fuel (Kawamura et al., 2012).
- There is a clear boundary of LG/MN ratios (∼10) between softwood and hardwood burning (Kawamura et al., 2012).
- Using LG/GA ratios can help to identify the burning of straws, woods, needles, and grasses, where most of the LG/GA ratios from burning of hardwood, straws, and grasses are higher than 10, quite different from those of needle plants.
- The measured LG/MN and LG/GA ratios in this study (Fig. 3) implied that the use of biofuels was mainly a mixture of softwood and crop straws.
- Compared with ratios in summer, LG/MN and LG/GA ratios in winter have a narrower range at both IAP and PG sites, and most of them converge at values lower than 10, suggesting wood burning may be dominant in winter, and the contribution of straw burning increases in summer.
- In addition, coal combustion may also emit LG, accounting for about 7% of LG in Beijing (Yan et al., 2018; Y. X. Zhang et al., 2008). Considering that the reported LG/MN and LG/GA ratios of coal combustion (both are in the range of 5–10) are close to those in our measurements, there may be a contribution from coal burning to levoglucosan, but if this amounts to 7% of levoglucosan, the percentage contribution of biomass burning to OC in winter falls to 10.3±1.6% and 10.2±1.5% at IAP and PG, respectively.
- Primary fossil-derived OC is mainly from coal combustion and traffic emissions in China.
- Such calculation shows that POC from coal combustion (POCcc) dominated POC at both sites in winter and summer campaigns.
- POCf was the largest contributor to OC at both sites through winter and summer. Significant contributions of SOCf in the winter sampling period indicated a greater fraction of OCf from ageing and oxidation.
- The total SOC accounts for 50.0±12.3% and 42.0±11.7% of OC for IAP and PG site in winter, demonstrating the important role of secondary formation processes, especially at the urban site.
- The average contributions of OCck were 3.6±2.7% and 13.4±5.8% in winter for IAP and PG and 17.4±12.5% and 10.4±6.7% in summer, close to those estimated in previous studies (19±4 %; Zhang et al., 2017).
- In the winter sampling campaign at IAP, POCf was the biggest contributor to OC, followed by SOCf. Both of them were significantly enhanced during haze periods, while the non-fossil fractions, OCbb, OCck, and SOCnf, did not show much difference between haze and non-haze periods. This implies the haze pollution at IAP in winter was elevated by the accumulation of coal combustion and traffic emissions under favourable weather conditions. The formation of secondary OC associated with coal combustion and traffic emissions was increased during the haze period.
- In the winter campaign at PG, POCf and SOCf were the top two contributors to OC; however, the contribution of POCf and SOCf did not increase much in the haze period. In contrast, the fractions of SOCnf increased substantially on 3 and 4 December, on which days there were found to be open burning activities in surrounding areas (shown from the fire spots on Fig. S2). This shows that a large proportion of OCbb was transformed to secondary OC during the transport of biomass burning aerosols to the receptor sites.
- WINSOCf has usually been seen as a proxy for primary fossil-derived OC in many previous studies (Liu et al., 2016c; Miyazaki et al., 2006).
- The lower water solubility of SOCf and SOCnf in winter may be due to them originating from the less oxidized semi-volatile POC from wood burning and anthropogenic emission at low temperatures. Weak photochemical activity would also lead to the formation of less oxidized SOC, which is more water-insoluble.
- However, more pronounced WINSOCnf in OCbb was found in winter, especially at the rural site. It seems that more water-insoluble fractions were observed in primary OC (POCf, POCnf, OCbb, and OCck) at the rural site in both winter and summer. It implies the emitted primary OC at the rural site was probably fresher and hence less aged and oxidized. On the other hand, the source emission profile at rural sites may be different from urban sites, with more heavy-duty diesel trucks with a high content of water-insoluble OC emitted in rural areas.
总结与启发
- 冬季观测期间,乡村站点的PM2.5浓度、OC/PM2.5、生物质燃烧示踪物(左旋葡聚糖、甘露聚糖和半乳糖聚糖)浓度都略高于城市站点。
- 放射性碳 (14C) 分析可以量化化石燃料和非化石来源对碳质气溶胶的贡献,因为非化石来源包含较高的当代 14C 含量,而化石来源碳质气溶胶中不含 14C。
- 化石源有机碳(OC from fossil sources,OCf)主要来自煤炭燃烧和车辆排放,非化石源有机碳(OC from non-fossil sources,OCnf)主要来自生物质燃烧、生物源排放和烹饪。
- 冬季观测期间,化石源是有机碳的主要来源,OCf 在城市站点和乡村站点分别占总 OC 的 67.8±4.0% and 59.3±5.7% 。冬季乡村站点非化石源有机碳占比要高于城市站点。
- 夏季 OCnf 占比要高于冬季,可能是由于生物质燃烧和生物源排放增加,以及燃煤源的降低。
- 有机碳可分为水不溶性有机碳(water-insoluble OC, WINSOC)和水溶性有机碳(water-soluble OC, WSOC)。
- 无论化石和非化石源,冬季水不溶性有机碳都是主要的成分。冬季乡村站点化石源排放的水不溶性有机碳占总碳的36.2±4.7%,非化石源排放的水不溶性有机碳占总碳的20.8±3.4%。注意此处是对总碳的占比,总碳=有机碳+无机碳。
- 冬季水不溶性有机碳主要来自化石源。城市和乡村站点化石源贡献的水不溶性有机碳占所有水不溶性有机碳的72.6±3.6% 和 63.4±6.5% 。
- 冬季左旋葡聚糖(Levoglucosan,LG)的浓度高于夏季。无论冬季还是夏季,乡村站点的LG浓度都高于城市站点。此外,冬季乡村站点LG与PM2.5、OC、EC的相关性要优于城市站点。以上分析表明冬季乡村生物质燃烧对乡村地区 PM2.5 有着更显著的影响。
- 左旋葡聚糖(Levoglucosan,LG)与 甘露聚糖(Mannosan,MN)的比值 LG/MN,左旋葡聚糖(Levoglucosan,LG)与 半乳糖聚糖(galactosan,GA)的比值 LG/GA。比值分析可用于确定生物质燃料的特定种类。本研究中,主要的生物质燃料为软木和农作物秸秆,冬季以软木为主,夏季以秸秆为主。
- 通过计算,乡村站点生物质燃烧排放的有机碳(OCbb)要高于城市站点。生物质燃烧排放的有机碳 = 软木排放的有机碳 + 秸秆排放的有机碳。
- 燃煤同样可以产生左旋葡聚糖。根据以往研究,燃煤贡献的左旋葡聚糖约占7%。
- 有机碳分为一次有机碳(POC)和次生有机碳(SOC)。SOC 约占 OC 的一半,表明二次形成过程的重要性。
- 燃煤是的 POC 主要来源。燃煤导致二次有机碳在冬季贡献更高,尤其是在城市地区。冬季化石源排放的一次有机碳主要为水不溶性有机碳 (Liu et al., 2016c; Miyazaki et al., 2006)。
- 冬季较低的水溶性 SOC 可能是由于冬季低温下,软木和其他人为源排放的 POC 中的半挥发性物质有较少氧化。相对较弱的光氧化活动同样导致较少氧化的 SOC 。
- 乡村站点生物质燃烧排放的有机碳对二次水不溶性有机碳有明显的贡献。而且发现乡村站点排放的一次有机碳(化石源一次有机碳、非化石源一次有机碳、生物质燃烧源有机碳和烹饪源有机碳)中水不溶性有机碳占比较高。说明乡村地区排放的一次有机碳更为新鲜,经历了较少的老化和氧化。另一方面,乡村站点和城市站点的有机碳有着不同的来源,乡村站点可能有较多的重型柴油卡车,这些重型柴油卡车排放的有机碳中有较高含量的水不溶性有机碳。
- 水不溶性有机碳对二次有机碳的明显贡献已在法国 (Sciare et al., 2011) 和瑞士(Zhang et al., 2016)被报道。
扩展阅读
- Gelencsér-2007-JGR: 区别来自化石燃料燃烧、生物质燃烧、生物排放和二次有机气溶胶的方法
- Simoneit-1999-AE: 左旋葡聚糖作为生物质燃烧的特征示踪物
- Fu-2016-AE: 生物质燃烧、真菌孢子和生物 SOA 的分子标记